Most of us remember being four or five years old and feeling the excitement of learning to tie our shoes for the very first time. Something similar to that excitement surged through a group of researchers at the University of Manchester’s School of Chemistry earlier this year, the only difference being that their laces were half a nanometer in diameter—about ten-thousand times thinner than a human hair.
The team of scientists, led by David Leigh, synthesized the most intricate and tightest chemical knot ever made by stringing together 192 carbon, oxygen, nitrogen, and iron atoms into a continuous looped structure. While past researchers—including Jean-Pierre Sauvage, who recently shared the 2016 Nobel Prize in Chemistry for his work on interlocked molecules—have managed to create chemical knots with three and five crossings (trefoil and pentafoil, respectively), the Manchester team managed to create a structure with an unprecedented eight crossings (octafoil). This morphology lent their knot considerably more strength than its precursors, giving it the potential to inspire applications in a range of fields, spanning from materials science to biochemistry and pharmacological chemistry.
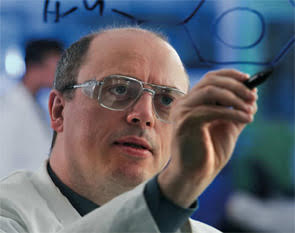
The key to accomplishing this chemical feat lies in a concept famous to physical organic chemists that is called “chemical preorganization” and dates back to Donald J. Cram, who many years ago shared the 1987 Nobel Prize in Chemistry. Within the Manchester team’s work, preorganization refers to the fact that, before attempting to actually form the new bonds that closed and tightened the knot, they had to perform a preliminary step that first weaved together the structure’s disparate strands, bringing them closer together before tying.
Though Sauvage and the other scientists who synthesized the earlier trefoil and pentafoil knots also relied on this fundamental chemical concept, they only successfully managed to weave together two macromolecular strands. “If you only tie two bits of string together, you can only make a very limited number of knots, whereas if you can braid three or maybe more strands, then you can, in principle, make billions of knots,” Leigh said. For Leigh and his coworkers, the key to successfully weaving together an unprecedented four macromolecular strands was to use four iron atom-supports, whose individual valence orbitals—which orient the atoms’ reactive electrons in three-dimensional space—were each perfectly shaped to coordinate two of the eight crossings. And the iron atoms did so well enough to lock all of the strands in place.
Next came the crux of the synthesis procedure: actually tying together the adjacent strands. To do this, the team relied on the fact that they had specifically imbued the individual strands with special reactivity in the form of double bonds—chemical entities inherently equipped with loosely held electrons, ready to react with one another and connect. Finally, the chemists isolated the final eight-fold knot, with a total unwound length of a miniscule twenty nanometers (500 times smaller than a red blood cell’s diameter), after removing the iron atom-supports in the final step.
In reflecting on his team’s achievement, Leigh jokingly remarked on the perspective of an outsider looking in. “It looks like it was just a stroke of magic or luck that after mixing together all the ingredients, it quickly assembled. But, in reality, synthesizing our knot was a bit like assembling a Boeing 747: the pieces won’t actually fit together unless you meticulously plan ahead of time,” Leigh said.
By far, though, the most exciting thing about the team’s invention is its endless applications. “Knotting and weaving have always led to breakthroughs in technology,” Leigh said. “In prehistoric times, humans wove knots to tie axe blades onto their handles. Technological improvements to weaving machines even helped spark the Industrial Revolution.”
Many foreseeable applications of Leigh’s knot also lay in the realm of materials and fabrics—within the field called materials science. Chemists and physicists might, for example, be able to knit together billions of these knots to engineer light, flexible, and durable materials. The fact that the team’s knot is so tight and strong might help it to eventually replace bulkier plastics and polymers like Kevlar—a material built from millions of tiny rod-like structures, densely aligned like boxed pencils—which is currently used to manufacture everything from bullet-proof vests to car breaks. Leigh explains this potential application by comparing sets of plate armor made from solid metal to sets made from interlocked metal chains. “Both can stop an arrow,” Leigh said. “The only difference is that one is much lighter and more flexible than the other. But does that really translate to the molecular level? We just don’t know.”
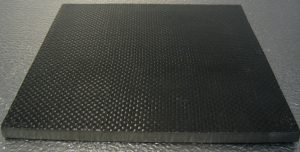
In addition to synthesizing their eight-fold knot, the Manchester team also demonstrated that it quickly forms a chemical complex, an association with nearby molecules, in solution with a negatively-charged chloride ion, whose volume fits perfectly within the knot’s open core. Such a discovery might allude to the knot motif’s potential applications in host-guest chemistry—an area in which organic chemists study the binding of different structures, usually of one or more small “guest” molecules and a macromolecular “host.” Scientists who study host-guest chemistry often work within biological and pharmacological contexts, trying to figure out ways to solubilize drugs and deliver them to targeted spots within our bodies, so as to minimize dosages. With Leigh and his team’s newfound method for synthesizing more intricate knots, those specializing in pharmacology may, for example, be able to incorporate knot motifs into hosts to lend them better structural complementarity to potential guests, improving current drug-delivery systems.
The team’s work may even help other groups of chemists synthesize molecules structurally unrelated to knots. Leigh and his team have previously shown that the simpler pentafoil knot can actually act as a catalyst, an agent that speeds up chemical reactions. But when they unwound that very same molecule, it ceased to be catalytically active. It’s truly astonishing to find that two molecules, each with the same connectivity, can behave so differently. Still, whether the team’s eight-fold knot also possesses this sort of marked catalytic activity hasn’t yet been verified.
With an eye towards the future, Leigh knows that many questions must still be answered before trying to apply their knot to real-world situations. How exactly does the knotted structure affect the strength of chemical bonds within? Does it make bonds weaker—a phenomenon we observe within the physical world, with fishing nets? If so, how much weaker? This amount of weakness might, for example, determine whether their structure stores enough energy in the form of strain to perform new chemical reactions, and whether this strain, on the flip side, would lessen their knot’s ability to replace strong materials like Kevlar.
At the current moment, the team members find themselves steeped in questions—many more than can currently be answered. And though the many potential applications tied up within their chemical knot currently hang in the realm of imagination, Leigh and his colleagues look forward to unraveling these possibilities in the near future.